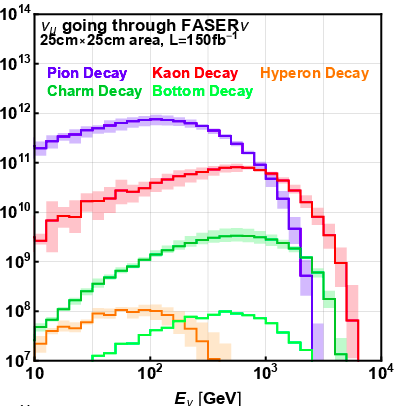
Since their discovery at a nuclear reactor in 1956, neutrinos have been detected from a variety of sources: beam dump experiments, cosmic ray interactions in the atmosphere, the Sun, the Earth, supernovae, and other astrophysical bodies outside our galaxy. The detection of neutrinos from these many sources has led to profound insights across the fields of particle physics, nuclear physics, and astrophysics.
At present, no neutrino produced at a particle collider has ever been detected. Colliders copiously produce both neutrinos and anti-neutrinos of all flavors and most are produced at very high energies where neutrino interactions are not well studied. Collider neutrinos are therefore highly complementary to those from other sources. Nevertheless, the highest energy collider neutrinos (and therefore the ones with the largest interaction cross sections) are produced along the beamline. Most collider detectors, however, have holes along the beamline to allow the passage of the beams, making them blind to this region. As a result, they miss the enormous flux of high-energy neutrinos streaming down the beam pipe. FASER, with its dedicated neutrino subdetector FASERν, covers this blind spot.
Collider neutrinos are predominantly produced in hadron decays. For an integrated luminosity of 150 fb−1 collected during LHC Run 3 at a center-of-mass energy of 14 TeV during 2021-2023, 2×1011 electron neutrinos, 6×1012 muon neutrinos, and 4×109 tau neutrinos (along with a comparable number of anti-neutrinos of each flavor) will stream through FASERν. For illustration, we show the spectrum of muon neutrinos going through FASERν in Fig. 1 separating the different processes contributing to neutrino production.
Assuming standard model cross sections, this implies that 1300 νe, 20 000 νμ, and 20 ντ will interact in FASERν. Notably, the mean energy of neutrinos that interact in FASERν is between 600 GeV and 1 TeV (depending on the flavor), and the corresponding spectrum of neutrinos scattering in the detector is shown in Fig. 2. These energies are above the energies of previously detected man-made neutrinos, but below the energies of the neutrinos detected by IceCube. This is shown in Fig. 3 for muon neutrinos and anti-neutrinos, where the expected uncertainties in the measurement of the corresponding neutrino-nucleon charged current (CC) cross section is illustrated.
FASERν will therefore not only detect the first collider neutrinos, but will also probe neutrino interactions in an energy range where neutrino cross sections are unconstrained. This will offer a host of interesting implications, such as bounding νμ interactions in the TeV energy range, adding significantly to the limited number of ντ events detected to date, detecting the highest energy man-made electron and tau neutrinos, and constraining the charm content of the nucleon. Furthermore, FASERν offers the prospect of opening up a new window to physics beyond the Standard Model (BSM).
The comparison of cross sections for three flavors will provide a test of lepton universality in neutrino scatterings. Recent results from collider experiments may suggest lepton universality violation in B-meson decays with a possible explanation by new, weak-scale physics. These flavor anomalies therefore motivate testing lepton universality in high-energy neutrino scattering, which may favor or exclude some of the proposed explanations.
More generally, it is also true that for many new physics scenarios, the BSM effects are most pronounced for heavy and third-generation fermions. This fact motivates probes of ντ properties (which are almost completely unconstrained) as well as νe and ντ production mechanisms (which receive significant contributions from charm and beauty hadron decays).
A more detailed discussion of the FASER Neutrino programme is given in the FASER's neutrino study.
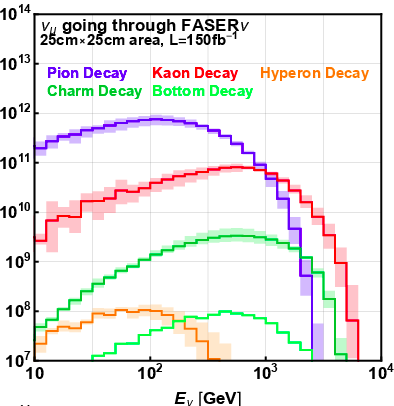
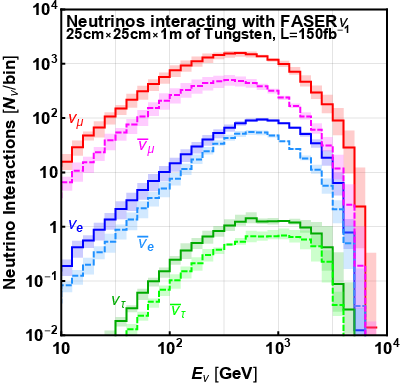
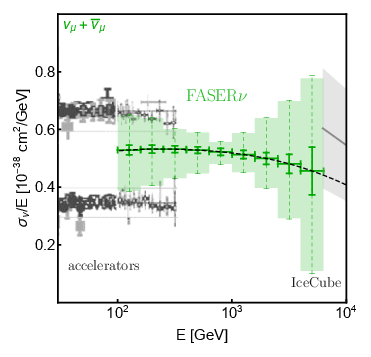